Introduction
The rotation of the Earth, with a period length of approximately 24 h, has been considered to induce the evolution within a large number of species referred to the circadian clock, which enables organisms to adapt their physiological and behavioral functions to anticipatory changes in the environment. Mammalian circadian clock machinery is hierarchically organized, consisting of the central clock in the suprachiasmatic nuclei (SCN) of the anterior hypothalamus, and subsidiary clocks in other brain regions and peripheral tissues. Both central and subsidiary clocks are governed by a self-sustained molecular oscillator consisting of a set of clock genes. The SCN entrains with the environmental light-dark cycle and then synchronizes subsidiary clocks through neurotransmitter and humoral factors; whereas subsidiary clocks in the periphery regulate tissue-specific functions in an anticipatory manner. Therefore, the circadian clock acts as a multifunction timer to regulate homeostatic systems, such as sleep and wakefulness, humoral secretions, immune system, and other functions, with a 24-h cycle. Such circadian variations in biological functions also affect the efficacy and toxicity of drugs: a large number of drugs cannot be expected to have the same potency at different administration times. Recent advances in our understanding of circadian biology and the development of available tools for characterizing the molecular clock reveal that choosing an appropriate dosing time improves the efficacy and safety of therapeutic drugs. Chrono-pharmaceutics is a research field that elucidates the circadian rhythm dependency of medications from the viewpoint of drug pharmacodynamics and pharmacokinetics. A variety of pathological conditions also exhibit day–night changes in symptom intensity, exemplified by allergic rhinitis, arthritis, asthma, myocardial infarction, congestive heart failure, stroke, and chronic pain disorders. Progression of research field of circadian biology enables to elucidate the underlying mechanism of circadian exacerbations in the pathological condition of various diseases. Currently, novel therapeutic approaches are facilitated by the development of chemical compounds targeted to an increasing number of key proteins that cause circadian exacerbation of pathological events. On the other hand, disruption of the circadian clock also leads to the onset of diseases. In particular, several epidemiological analyses and laboratory animal studies demonstrate that circadian rhythm disruption is associated with cancer development and tumorigenesis. This review presents an overview of the current understanding of the role of the circadian biological clock in regulating drug efficacy and disease conditions, especially focusing on cancer chemotherapy and pain relief, and also describes the importance of identifying the difference in the circadian machinery between diurnal and nocturnal animals to select the most appropriate times of day to administer drugs for disease treatment.
Molecular mechanism of the mammalian circadian clock
In mammals, the circadian clock machinery consists of a hierarchical assembly of multiple endogenous oscillators. Among these oscillators, a major pacemaker is located in the SCN of the hypothalamus.
The suprachiasmatic circadian clock is entrained to a 24-h period by daily light input from the visual neural system and produces output signals for coordinating the phase of the subsidiary oscillators in other brain regions and peripheral tissues. Circadian oscillations in both suprachiasmatic and subsidiary clocks are driven by interconnected transcriptional and translational feedback loops, consisting of clock genes. The gene products of Clock and Bmal1 (also known as Arntl) form a heterodimer that functions as a positive regulator to activate the transcription of the Period (Per) and cryptochrome (Cry) genes. Once the PER and CRY proteins reach a critical concentration, they act as negative transcription factors to attenuate CLOCK/BMAL1- mediated transactivation. Rev-erbα (known as Nrd1d1) is also activated by CLOCK/BMAL1 and transrepressed by PER and CRY, resulting in circadian oscillation in the expression of REV-ERBα. In turn, REV-ERBα periodically represses Bmal1 transcription, thereby interconnecting the positive and negative loops (Figure 1 A). Similar to the mechanism of Rev-erbα transcription, the products of clock genes, comprising the core oscillation loop, also control daily variations in output physiology through periodic activation/repression of clock-controlled output genes. Activating transcription factor-4 (ATF4) and proline- and acid-rich (PAR) basic Leucine zipper (bZIP) proteins, hepatic leukemia factor (HLF), thyrotroph embryonic factor (TEF), and D-site binding protein (DBP) are examples of such output mediators because their expression is regulated by core oscillator components28–30) (Figure 1 B). The circadian-controlled output pathways include those that control the expression of many enzymes and regulators involved in drug sensitivity and xenobiotic detoxification.
As the expression of up to 10% of genes has been suggested to be under the control of the circadian clock, it is not surprising that the circadian clock system also affects the pathological condition and/or the onset of diseases.
Circadian clock regulation of chemosensitivity of cancer cells
There is accumulating evidence that circadian clock disruption is associated with cancer development and tumorigenesis however, the sensitivity of cancer cells to chemotherapeutic agents is reported to exhibit dosing time dependent variations. Tumor masses are composed of heterogeneous cells, and this heterogeneity is related to resistance to chemotherapy and a high risk of recurrence. Cancer stem-like cells (CSCs) represent a distinct proportion in tumor masses, but they play a critical role in driving tumor growth, progression, and metastasis due to their self-renewal and differentiation capacities. The tumor microenvironment surrounding CSCs is also responsible for malignant properties.
These malignant properties are thought to be caused at the molecular level, and interaction of CSCs with the microenvironment also contributes to tumor malignancy. Molecular circadian oscillators consisting of clock genes induce the time-dependent changes in the chemosensitivity of cancer cells by controlling the expression of cell cycle regulators and apoptotic factors. However, recent studies demonstrate that oscillation in the expression of clock genes is disrupted in CSCs, but not in the surrounding microenvironment cells, suggesting a distinct role of the circadian clock between CSCs and non-CSCs. The pathological significance of circadian clock disruption in CSCs is not fully understood, but oncogenic transformation of circadian gene Per2-defective (Per2m/m) fibroblasts renders them resistant to common chemotherapeutic drugs, accompanying up-regulation of the aldehyde dehydrogenase 3a1 (Aldh3a1) gene.50) Co-expression of the oncogenes H-rasV12 and SV40 large T-antigen induces malignant transformation of Per2m/m cells, characterized by increased spheroid formation and tumor growth. The cytotoxic effects of the chemotherapeutic agents, methotrexate, gemcitabine, etoposide, vincristine, and oxaliplatin, are also significantly attenuated in the oncogenic Per2m/m cells. The increased levels of ALDH3A1 in the oncogenic Per2m/m cells prevent chemotherapeutic druginduced accumulation of reactive oxygen species (Figure 2 A). The expression levels of known cancer stemness markers Klf4, Pou5f1, and c-Myc also increased in Per2m/m cells after oncogenic transformation, suggesting the relationship of circadian clock disruption with CSCs properties. In contrast to CSCs, the expression of clock genes in the tumor microenvironment including non-CSCs exhibits significant circadian oscillation, suggesting the underlying mechanism of dosing time-dependent difference in the chemosensitivity of tumors. Common chemotherapeutic agents prevent DNA synthesis and cell division, and then ultimately cause cell death through cell-intrinsic pathways. The p53 tumor suppressor gene (TRP53) plays a critical role in the regulation of cell death induced by chemotherapeutic agents, but p53 protein abundance in tumor cells exhibits circadian oscillation. ATF4, a component of the mammalian circadian clock, functions in the circadian accumulation of p53 protein in tumors. In murine fibroblast tumors, ATF4 induces the circadian expression of p19ARF (also known as Cdkn2). Oscillation of p19ARF interacts in a time-dependent manner with MDM2, a specific ubiquitin ligase of p53, resulting in the rhythmic prevention of its degradation by MDM2. Consequently, the half-life of p53 protein varies in a circadian time-dependent manner without variation in mRNA levels. The p53 protein accumulates during the times of day when the p19ARF–MDM2 interaction is facilitated, and the anti-tumor effects of chemotherapeutic agents are promoted by administering them during p53 accumulation (Figure 2 B). As such, the role of the circadian clock in the regulation of chemosensitivity is different between CSCs and non-CSCs. Novel therapeutic approaches are required to treat cancers and eradicate CSCs.
Role of the circadian clock in the physiopathology of neuropathic pain
Pain is a common problem in cancer patients when tumors or their metastases grow in the proximity of nerves. Around 40% of cancer patients have pain that includes a neuropathic component and 30% of such pain is directly related to tumor involvement of nervous tissue. This cancer-induced neuropathic pain is generally believed to be due to mechanical nerve compression by tumors or cancer cell infiltration into the nerve. One troublesome hallmark symptom of neuropathic pain is hypersensitivity even evoked by a simple touch, pressure from clothing or gentle massage, which is also known as ‘mechanical allodynia.’ After peripheral nerve injury, the number of microglia is increased in the dorsal horn area of the spinal cord and a specific type of purinergic receptors are expressed on microglia. Extracellular ATP stimulates purinergic receptors on activated microglia, resulting in the release of proinflammatory cytokines, chemokines, and trophic factors. Stimulation of purinergic receptors on activated microglia by ATP induces the release of brain-derived neurotrophic factor (BDNF), and then the neurotrophic factor acts on TrkB in lamina I neurons, causing an altered transmembrane anion gradient by down regulating K+-Cl− co transporter 2 (KCC2).
Down regulation of KCC2 changes γ-amino butyric acid (GABA)- and glycine-evoked responses from inhibitory to excitatory, which induces mechanical pain hypersensitivity in response to normally innocuous stimuli? Circadian alterations in pain hypersensitivity have also been confirmed in patients with cancer, rheumatoid diabetic neuropathy, fibromyalgia, and multiple sclerosis, but the underlying mechanism is not well understood. Partial sciatic nerve ligation (PSL) is a well-established nerve injury model that produces pain hypersensitivity in laboratory animals. Neuropathic pain hypersensitivity in PSL mice also exhibits marked circadian alterations, which depend on the circadian secretion of glucocorticoids from the adrenal glands Circadian exacerbation of pain hypersensitivity is mediated by glucocorticoid-induced increases in the extracellular release of ATP in the spinal cord, which stimulates purinergic receptors on microglia in the dorsal horn. Serum- and glucocorticoid-inducible kinase-1 (SGK-1) is the key molecule responsible for the glucocorticoid-enhanced release of ATP from astrocytes. SGK-1 protein levels in spinal astrocytes increase in response to glucocorticoid stimuli and promote ATP release by opening the pannexin-1 hemichannels (Figure 3). As adrenal glucocorticoid secretion is regulated by products of clock genes, the molecular circadian machinery also affects neuropathic pain hypersensitivity. Although inhibition of SGK-1 activity alleviates neuropathic pain hypersensitivity, there are no available strategies to inhibit SGK-1 in the spinal cord. As the result of screening a clinically approved drug library, sulfasalazine is identified as having inhibitory effects on SGK-1.62) Sulfasalazine is a prodrug composed of 5-aminosalicylic acid and sulfapyridine linked by an N=N bond, which is therapeutically effective against inflammatory bowel diseases and rheumatoid arthritis. However,
Figure 4
Difference in the rhythmic phase of clock genes and p-gp in peripheral tissue between nocturnally active rodents and diurnally active monkey
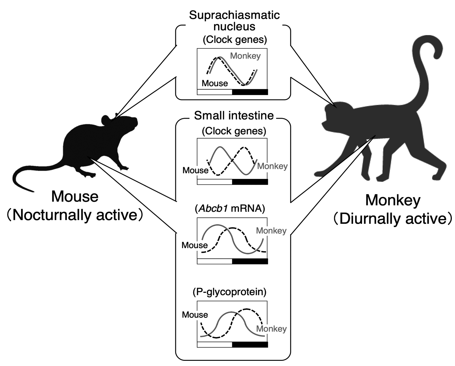
Neither 5-aminosalicylic acid nor sulfapyridine has a negligible effect on the SGK-1 activity, implying that the N=N bond in sulfasalazine is necessary for its inhibitory action against SGK-1. After intrathecal injection to PSL mice, sulfasalazine alleviates pain hypersensitivity. On the other hand, no significant anti-neuropathic pain effects are observed after oral administration of sulfasalazine due to its low bioavailability and limited spinal distribution, which are caused by the xenobiotic transporter breast cancer resistance protein (BCRP). Concomitant oral administration of sulfasalazine with febuxostat, which is an approved drug to inhibit BCRP, improves the distribution to the spinal cord. Concomitant oral administration with febuxostat also enables sulfasalazine to exhibit the anti-neuropathic pain effects on PSL mice. Interestingly, the anti-neuropathic pain effects of sulfasalazine are observed at the times of day when spinal expression of SGK-1 is increased. This also indicates that concomitant oral administration of sulfasalazine with febuxostat may also be a preventative option for circadian exacerbation of neuropathic pain hypersensitivity.
Species-dependent difference in the circadian clock-associated xenobiotic detoxification
Dosing time-dependent alterations in the effectiveness and toxicity of drugs are associated not only with the circadian variations in the susceptibility to drugs, but also with its pharmacokinetics.8,13) Recent studies using laboratory rodents demonstrate that circadian oscillation in the physiological functions affecting absorption, distribution, metabolism and excretion of drugs, which underlies the cause of the dosing time-dependent changes in pharmacokinetics. However, it is difficult to predict the circadian change in the drug pharmacokinetics in a diurnal human using the data collected from nocturnal rodents. Most laboratory rodents are active at night, but humans exhibit higher activity during the daytime. Although the fundamental mechanism of the circadian clock system is conserved beyond the species, the timekeeping system in nocturnal animals is not simply inverted relative to that in diurnal species. Therefore, recognition of the difference in the rhythmicity between diurnal and nocturnal animals is important for prediction of the dosing time-dependency of drug disposition. The rhythmic phase of circadian gene expression in the SCN of cynomolgus monkeys, which are diurnally active animals as similar to humans, is not significantly different from that reported previously in nocturnal rodents. However, the oscillation in the expression of circadian clock genes and clock-controlled genes in the peripheral tissues of monkeys exhibits at opposite phases of those observed in rodents. The xenobiotic transporter P-glycoprotein (P-gp), encoded by ATP-binding cassette transporter gene Abcb1, functions as efflux pump by expelling cytotoxic substances from cells. In mammals, P-gp is expressed in the epithelial cells of liver, intestine, and kidney, and thus functions in the biliary, intestinal, and renal elimination of many drugs. P-gp also functions as an intestinal barrier to restrict oral drug absorption. Intestinal expression of P-gp exhibits significant circadian oscillation in both nocturnally active rodents and diurnally active monkeys. The oscillation in the intestinal expression of P-gp affects the pharmacokinetics of its substrates. The expression of P-gp in the small intestine is under the control of the molecular circadian clock, but the rhythmic pattern of P-gp expression in diurnally active monkeys is substantially different from that in nocturnally active rodents (Figure 4). Although it remains to be clarified why the rhythmic phase in the intestinal expression of clock genes and xenobiotic transporters is different between diurnal and nocturnal species, intestinal expression of P-gp in both species increases before their daily feeding time. As P-gp recognizes a broad range of substances, including not only medical drugs but also harmful compounds, circadian clock machinery may function to protect animals against harmful compounds contained in foods by increasing the expression of P-gp before feeding. Such a mechanism is beyond the species, resulting in the cause of dosing time-dependent changes in the intestinal absorption of drugs. Identification of factors reflecting the circadian changes in the drug disposition in laboratory monkeys may improve the predictive accuracy of pharmacokinetics in humans.
Conclusion
The individualization of pharmacotherapy has been conducted by observation of the drug concentration in blood, and then dosage adjustment is performed based on interindividual differences in drug pharmacokinetics. However, in addition to interindividual differences, intraindividual variations should be considered to further improve rational pharmacotherapy because the susceptibility to drugs and their disposition are changed according the times of daty. The effectiveness and toxicity of drugs, including chemotherapeutic agents, also vary with the circadian changes in the cellular sensitivity and activity of target molecules. However, many drugs are still administered without consideration of the time of day. Identification of the mechanism underlying the circadian alterations of drug pharmacokinetics and pharmacodynamics will help to achieve better pharmacotherapy for disease treatment. Acknowledgments This work was supported in part by a Grant-in-Aid for Scientific Research B (15H04765), by a Grant-in-Aid for Scientific Research A (18H04019), by Challenging Exploratory Research (17K19493 and 20K21484) and the Platform Project for Supporting Drug Discovery, and Life Science Research [Basis for Supporting Innovative Drug Discovery and Life Science Research (BINDS) from AMED (Grant No. JP20am0101091). I would like to express my thanks to Dr. Yuya Yoshida for his support in the preparation of graphical abstract. Conflict of Interest The author declares no conflict of interest.